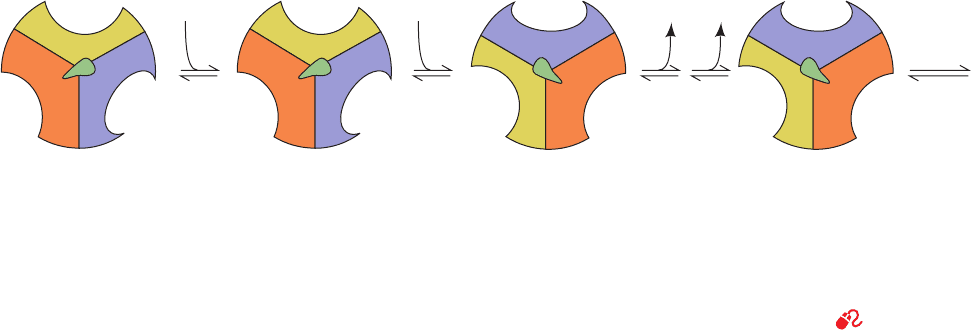
the ATP-containing T site to an “open” (O) site and con-
vert the O site to an L site.
3. ATP is synthesized at the T site on one subunit while
ATP dissociates from the O site on another subunit. On the
surface of the active site, the formation of ATP from ADP
and P
i
entails little free energy change, that is, the reaction
is essentially at equilibrium. Consequently, the free energy
supplied by the proton flow primarily facilitates the release
of the newly synthesized ATP from the enzyme; that is, it
drives the T S O transition, thereby disrupting the
enzyme–ATP interactions that had previously promoted
the spontaneous formation of ATP from ADP ⫹ P
i
in the
T site.
How is the free energy of proton transfer coupled to
the synthesis of ATP? Boyer proposed that the binding
changes are driven by the rotation of the catalytic assembly,
␣
3

3
with respect to other portions of the F
1
F
0
–ATPase.This
hypothesis is supported by the X-ray structure of F
1
.Thus,
the closely fitting nearly circular arrangement of the ␣
and  subunits’ inner surface about the ␥ subunit’s helical
C-terminus is reminiscent of a cylindrical bearing rotating
in a sleeve (Fig. 22-38c). Indeed, the contacting hydropho-
bic surfaces in this assembly are devoid of the hydrogen
bonding and ionic interactions that would interfere with
their free rotation; that is, the bearing and sleeve appear
to be “lubricated.” Moreover, the central cavity in the
␣
3

3
assembly (Fig. 22-38a) would permit the passage of
the ␥ subunit’s N-terminal helix within the core of this
particle during rotation. Finally, the conformational dif-
ferences between F
1
’s three catalytic sites appear to be
correlated with the rotational position of the ␥ subunit.
Apparently the ␥ subunit, which is thought to rotate within
the fixed ␣
3

3
assembly, acts as a molecular cam shaft in
linking the proton-motive force–driven rotational motor to
the conformational changes in the catalytic sites of F
1
.This
concept is also supported by molecular dynamics simula-
tions (Section 9-4) by Leslie, Walker, and Martin Karplus,
which indicate that the conformational changes in the 
subunits arise from both steric and electrostatic interac-
tions with the rotating ␥ subunit.
Rotating assemblies are not unprecedented in biologi-
cal systems. Bacterial flagella, which function as propellers,
had previously been shown to be membrane-mounted ro-
tary engines that are driven by the discharge of a proton
gradient (Section 35-3Ib).
e. The F
1
F
0
–ATPase Is a Rotary Engine
In the F
1
F
0
–ATPase, the rotor is proposed to be an as-
sembly of the c ring and its associated ␥ and (E. coli) ε sub-
units, whereas the ab
2
unit and the (E. coli) ␦ subunit to-
gether with the ␣
3

3
spheroid form the stator (Fig. 22-41).
The rotation of the c ring in the membrane relative to the
stationary a subunit is driven by the migration of protons
from the outside to the inside, as we discuss below. The pe-
ripheral arm (b
2
␦) presumably functions to hold the ␣
3

3
spheroid in place while the ␥ subunit rotates inside it.
The rotation of the E. coli ␥ε–c-ring rotor with respect
to the ab
2
␦–␣
3

3
stator has been ingeniously demonstrated
by Masamitsu Futai using techniques developed by
Kazuhiko Kinosita Jr. and Masasuke Yoshida (Fig. 22-43a).
The ␣
3

3
spheroid of E. coli F
1
F
0
–ATPase was fixed, head
down, to a glass surface as follows: Six consecutive His
residues (a so-called His Tag; Section 6-3Dg) were muta-
genically appended to the N-terminus of the ␣ subunit,which
is located at the top of the ␣
3

3
spheroid as it is drawn in
Fig. 22-38a. The His-tagged assembly was applied to a glass
surface coated with horseradish peroxidase (which, like most
proteins, sticks to glass) conjugated with Ni
2⫹
-nitriloacetic
acid [N(CH
2
COOH)
3
, which tightly binds His tags], thereby
binding the F
1
F
0
–ATPase with its F
0
side facing away from
the surface. The Glu 2 residues of this assembly’s c sub-
units, which are located on the side of the c ring facing away
from F
1
, had been mutagenically replaced by Cys residues,
which were then covalently linked to biotin (a coenzyme
that normally participates in carboxylation reactions; Sec-
tion 23-1Ab). A fluorescently labeled and biotinylated
(at one end) filament of the muscle protein actin (Section
35-3Ac) was then attached to the c subunit through the ad-
dition of a bridging molecule of streptavidin, a protein that
avidly binds biotin to each of four binding sites (Cys 193 of
Section 22-3. Oxidative Phosphorylation 857
Figure 22-42 Energy-dependent binding change mechanism
for ATP synthesis by proton-translocating ATP synthase. F
1
has
three chemically identical but conformationally distinct
interacting ␣ protomers: O, the open conformation, has very
low affinity for ligands and is catalytically inactive; L has loose
binding for ligands and is catalytically inactive;T has tight
binding for ligands and is catalytically active. ATP synthesis
occurs in three steps. (1) Binding of ADP and P
i
to site L.
(2) Energy-dependent conformational change converting
12
L
T
ADP + P
i
Energy
3
ATP H
2
O
ADP • P
i
ADP • P
i
ATP
ATP
ATP
O
L
T O
T
O L
T
ATP
O L
binding site L to T,T to O, and O to L. (3) Synthesis of ATP at
site T and release of ATP from site O. The enzyme returns to its
initial state after two more passes of this reaction sequence. The
energy that drives the conformational change is apparently
transmitted to the catalytic ␣
3

3
assembly via the rotation of the
␥ε assembly (in E. coli; ␥␦ε in mitochondria), here represented
by the centrally located asymmetric pointer (green). [After Cross,
R.L., Annu. Rev. Biochem. 50, 687 (1980).]
See the Animated
Figures
JWCL281_c22_823-870.qxd 7/20/10 6:25 PM Page 857