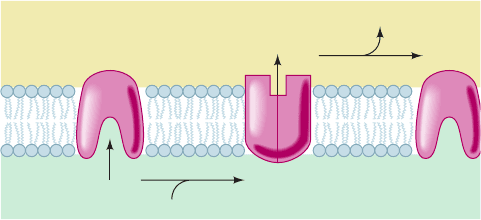
rotation about a bond connecting the semiquinone ring to its
nonpolar tail), which it then reduces. Thus, is unable to
reduce the ISP (after it has reduced cytochrome c
1
) be-
cause it is too far away to do so. This novel mechanism is
supported by the observation that mutagenically inserting
a disulfide bond either within the hinge of the ISP or be-
tween it and cytochrome b greatly diminishes the activity
of Complex III but that its activity is restored on exposure
to reducing agents.
g. The Proton Pump Mechanism
Complex IV (COX) transports four protons from the
matrix to the intermembrane space for each O
2
it reduces
(2H
⫹
per electron pair; Fig. 22-14). It contains no (H
⫹
⫹ e
⫺
)
carriers and hence cannot do so via a redox loop (Q cycle-
like) system. Rather, as we shall see, it does so via the pro-
ton pump mechanism (Fig. 22-33), which does not require
that the redox centers themselves be H
⫹
carriers. In this
model, the transfer of electrons results in conformational
changes to the complex. The unidirectional translocation of
protons occurs as a result of the influence of these confor-
mational changes on the pK’s of amino acid side chains and
their alternate exposure to the internal and external side of
the membrane. We have previously seen that conformation
can influence pK. The Bohr effect in hemoglobin, for ex-
ample, results from conformational changes induced by O
2
binding, which causes pK changes in protein acid–base
groups (Section 10-2E). If such a protein were located in a
membrane and if, in addition to pK changes, the conforma-
tional changes altered the side of the membrane to which
the affected amino acid side chains were exposed, the re-
sult would be H
⫹
transport and the system would be a
proton pump.
Keep in mind that protons, being atomic nuclei, must al-
ways be associated with molecules or ions. Consequently, a
proton cannot be transported across a membrane in the
same way that, say, a K
⫹
ion is. Rather, protons are translo-
cated by hopping along chains of hydrogen bonded groups
Q
⫺
ⴢ
in the transport protein in much the same way that hydro-
nium ions migrate through an aqueous solution (Fig. 2-10),
that is, they move along a proton wire (Section 21-2Al).
However, unlike a wire in an electrical circuit, all elements
of a proton wire need not be connected at the same time
and, moreover, internal water molecules, which are not al-
ways apparent in protein X-ray structures, are likely to be
integral parts of the proton wire. Hence, elucidating the
precise pathway of proton transport through a protein is a
difficult and uncertain task. Note that proton pumps, like
other active transport systems, must be gated to prevent
protons from leaking back through the pump and thus
short-circuiting the system.
h. Bacteriorhodopsin Is a Light-Driven Proton Pump
The simplest known and best characterized proton
pump is the intrinsic membrane protein bacteriorhodopsin
of Halobacterium halobium. It consists mainly of seven
transmembrane helices, A through G, that form a central
polar channel (Section 12-3Ab). This channel contains a
retinal prosthetic group that is covalently linked, via a pro-
tonated Schiff base, to Lys 216, which extends from helix G
(Fig. 12-24). The protein obtains the free energy required
for unidirectionally pumping protons from the absorption
of a photon by the retinal. This initiates a sequence of
events in which the protein conformationally adjusts
through successive spectroscopically characterized inter-
mediates, designated J, K, L, M, N, and O, as the system de-
cays back to its ground state over a period of ⬃10 ms. The
result of this cycle is the net translocation of one proton
from the cytoplasm to the extracellular medium, thereby
converting light energy to proton-motive force. The as yet
incompletely understood mechanism of this process, which
was elucidated through detailed structural, mutational, and
time-resolved spectroscopic studies carried out in several
laboratories, is outlined in Fig. 22-34:
1. On absorbing a photon, the ground state all-trans-
retinal photoisomerizes to its 13-cis form. This is a multi-
step process that rapidly passes (in ⬃3 ps) through the J
and K states. The free end of the retinal, which is now
twisted around the newly cis double bond, moves relative
to the protein scaffold such that retinal’s C13 methyl group
and its C14 atom shift toward the inside by 1.3 and 1.7 Å,
respectively. This yields the L state.
2. Further conformational adjustments yield the M
state. Here the N atom of the Schiff base has rotated and
shifted from its ground state position, in which it is hydro-
gen bonded to an internal water molecule, to one in which
it points toward the inside face of the protein in the vicinity
of the hydrophobic side chains of Val 49 and Leu 93. This
reduces the pK of the protonated Schiff base. In contrast,
the pK of Asp 85 increases. This is because, in the ground
state,Asp 85 effectively serves as the counterion of the pro-
tonated Schiff base and participates in a hydrogen bonded
network with three internal water molecules, but in the M
state, it is only associated with a single water molecule.
Consequently, the Schiff base protonates Asp 85. This
process is facilitated by a slight movement of helix C that
850 Chapter 22. Electron Transport and Oxidative Phosphorylation
Figure 22-33 Proton pump mechanism of electron
transport–linked proton translocation. At each H
⫹
translocation
site, n protons bind to amino acid side chains on the matrix side
(inside) of the membrane. Reduction causes a conformational
change that decreases the pK’s of these side chains and exposes
them to the cytosolic side (outside) of the membrane, where the
protons dissociate. Reoxidation results in a conformational
change that restores the pump to its original conformation.
nH
+
nH
+
e
–
e
–
Intermembrane
space
Matrix
reduction
reoxidation
JWCL281_c22_823-870.qxd 6/8/10 9:19 AM Page 850