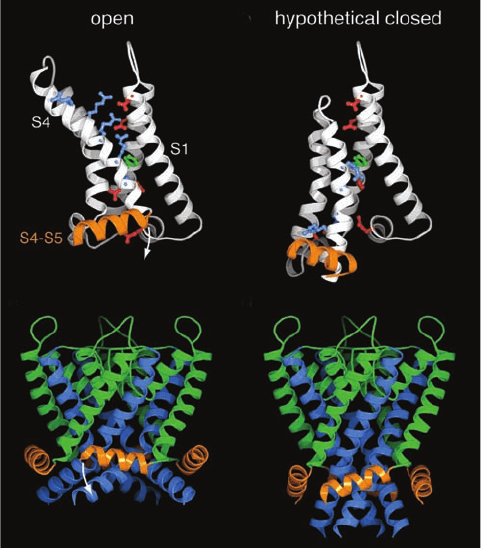
later, a phenomenon that has important consequences for
the transmission of nerve impulses (Section 20-5B). In fact,
minute “gating currents” arising from the movements of
these positively charged gates in opening and closing can
be detected (electrical current is the movement of charge)
if the much larger currents of K
⫹
ions through the mem-
brane are first blocked by plugging the Kv channel from its
cytosolic side by high concentrations of Cs
⫹
or tetraethyl-
ammonium ions (which are too large to pass through the
K
⫹
pore but apparently become stuck within it).
c. Inactivation Occurs through the Insertion of the Kv
Channel’s N-Terminal Peptide into Its Central Pore
The inactivation of the Kv channel is abolished by pro-
teolytically excising its N-terminal ⬃20-residue segment
(its inactivation peptide), which NMR studies indicate
forms a ball-like structure. However, when chemically syn-
thesized inactivation peptide is injected into the cytosol,
the truncated Kv channel inactivates at a rate proportional
to the concentration of inactivation peptide. This suggests
that inactivation occurs when the inactivation ball swings
around at the end of the ⬃65-residue peptide linking it to
the T1 domain so as bind to the open K
⫹
pore in a way that
physically blocks the passage of K
⫹
ions—the so-called
ball-and-chain mechanism. Indeed, the time the Kv chan-
nel stays open varies with the length of this “chain,” as mu-
tationally adjusted.
Where is the inactivation peptide’s binding site? Muta-
tional analysis by MacKinnon revealed that the hydropho-
bic residues lining the Kv channel’s internal pore and cen-
tral cavity form the receptor site for the inactivation
peptide. Since the ⬃6-Å-diameter cytosolic entrance to the
internal pore is too narrow to admit the ball, the ball pep-
tide must unfold in order to enter the internal pore. The
first 10 residues of ball peptides are predominantly hy-
drophobic, whereas the succeeding 10 residues are largely
hydrophilic and contain several basic residues. It therefore
appears that inactivation occurs through the binding of the
N-terminus of the fully extended inactivation peptide in-
side the internal pore via hydrophobic interactions, an as-
sociation that is augmented by the binding of the basic
residues in the ball peptide’s C-terminal segment to the
acidic residues lining the entrance of the internal pore.
Thus, the inactivation peptide acts more like a snake than a
ball and chain.
How does the inactivation peptide gain access to the inter-
nal pore, which appears to be covered by the T1 tetramer?
The passage through the center of the T1 tetramer, as seen
in its X-ray structure (Fig. 20-33b), is too narrow to permit
the passage of the inactivation peptide. Moreover, Christo-
pher Miller eliminated the possibility that the individual T1
domains in a tetramer can ever separate far enough to ad-
mit the inactivation peptide by showing that cross-linking
adjacent T1 domains by genetically engineered disulfide
bonds (whose positions were selected by referring to the
T1 X-ray structure) does not significantly affect the Kv
channel’s gating properties. This strongly suggests that the
inactivation peptide gains access to the bottom of the
transmembrane pore through lateral windows between the
transmembrane and T1 domains, whose sides are formed
by the ⬃35-residue peptide segment linking these domains.
Presumably, K
⫹
ions pass through these same windows
when the Kv channel is open.
A Kv channel engineered so that only one subunit has
an inactivation peptide still inactivates but at one-fourth
the rate of normal Kv channels. Apparently, any of the nor-
mal Kv channel’s four inactivation peptides can block the
774 Chapter 20. Transport Through Membranes
(a)
(c)
(d)
(b)
Figure 20-34 Proposed mechanism of voltage gating in the
Kv1.2 voltage-gated K
⫹
channel. (a) The voltage-sensing domain
in the X-ray structure of Kv1.2 in its open conformation as
viewed from the pore parallel to the plane of the membrane with
the extracellular solution above. The polypeptide chain is drawn
in ribbon form with helices S1 through S4 white and the S4–S5
linker helix orange. Side chains are shown in ball-and-stick form
with those of the Arg and Lys residues of S4, the so-called gating
charges, cyan, those of Asp and Glu in the so-called external and
internal clusters red, and that of Phe 233, which separates the
external and internal clusters, green. Note that the gating charges
extend upward into the extracellular solution and associate with
the external cluster. (b) The voltage-sensing domain in the
hypothetical closed conformation displayed as in Part a. S4,
together with S3, has moved downward relative to S1 and S2
toward the now more negatively charged cytosol, thereby
pivoting the S4-S5 helix in the direction of the white arrow in
Part a. Here the gating charges extend downward toward the
cytosol and associate with the internal cluster. (c) The S4–S6
linkers and the K
⫹
pore assembly, which includes helices S5 and
S6, in the X-ray structure of Kv1.2 as viewed in the opposite
direction from Part a.The S6 helices are blue, the S4–S5 linkers,
which contact S6, are orange, and the remainder of the pore
assembly is green. (d) Hypothetical model of the S4–S5 linker in
the closed conformation, displayed as in Part c, with the structure
of the K
⫹
pore assembly based on the X-ray structure of the
KscA channel (Fig. 20-12a). [Courtesy of Roderick MacKinnon,
Rockefeller University. PDBid 2R9R.]
JWCL281_c20_744-788.qxd 7/1/10 7:58 AM Page 774