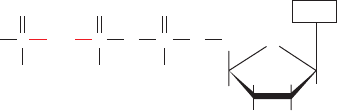
influence termination efficiency. Indeed, mutations in the 
subunit of RNAP can both increase and decrease termina-
tion efficiency. However, the way in which hairpin forma-
tion induces allosteric changes to RNAP is as yet unknown.
b. Many Bacterial Terminators Require the
Assistance of Rho Factor
Around half the termination sites in E. coli lack any ob-
vious similarities and are unable to form strong hairpins;
they require the participation of a protein known as Rho
factor to terminate transcription. Rho factor was discovered
through the observation that in vivo transcripts are often
shorter than the corresponding in vitro transcripts. Rho
factor, a RecA family hexameric helicase (Section 30-2Ca)
of identical 419-residue subunits, enhances the termination
efficiency of spontaneously terminating transcripts as well
as inducing the termination of nonspontaneously terminat-
ing transcripts.
Several key observations have led to a model of Rho-
dependent termination:
1. Rho unwinds RNA–DNA and RNA–RNA double
helices by translocating along a single strand of RNA in its
5¿S3¿ direction.This process is powered by the hydrolysis
of NTPs to NDPs ⫹ P
i
with little preference for the iden-
tity of the base. NTPase activity is required for Rho-
dependent termination as is demonstrated by its in vitro
inhibition when the NTPs are replaced by their ,␥-imido
analogs,
substances that are RNAP substrates but cannot be hy-
drolyzed by Rho.
2. Genetic manipulations indicate that Rho-dependent
termination requires the presence of a specific recognition
sequence on the newly transcribed RNA upstream of the
termination site. The recognition sequence must be on the
nascent RNA rather than the DNA as is demonstrated by
Rho’s inability to terminate transcription in the presence
of pancreatic RNase A. The essential features of this ter-
mination site have not been fully elucidated; the construc-
tion of synthetic termination sites indicates that it consists
of 80 to 100 nucleotides that lack a stable secondary struc-
ture and contain multiple regions that are rich in C and
poor in G.
These observations suggest that Rho attaches to nascent
RNA at its recognition sequence [named rut (for Rho util-
ization), a C-rich segment of at least 40 nt] and then
translocates along the RNA in the 5¿S3¿ direction until it
encounters an RNAP paused at the termination site (with-
out the pause, Rho might not be able to overtake the RNA
,␥-Imido nucleoside triphosphate
O
HH
HH
OHOH
CH
2
OO
O
O
ⴚ
O
ⴚ
PP
Base
O
O
ⴚ
PNH
O
O
ⴚ
polymerase). There, as Jeffrey Roberts has shown, Rho
pushes the RNAP forward in a way that partially rewinds
its dsDNA helix at the transcription bubble while unwind-
ing the RNA–DNA hybrid helix (forward translocation),
thus releasing the RNA. Rho-terminated transcripts have
3¿ ends that typically vary over a range of ⬃50 nucleotides.
This suggests that Rho pries the RNA away from the tem-
plate DNA rather than “pushing” an RNA release “but-
ton.” TCRF (alternatively, Mfd), which functions during
transcription-coupled repair in E. coli to release a stalled
RNAP from a damaged template by stripping away its
bound RNA (Section 30-5Bb), is an ATP-powered DNA
translocase that is thought to mechanically act on RNAP in
much the same way as Rho.
Each Rho subunit consists of two domains that can be
separated by proteolysis: Its N-terminal domain binds
single-stranded polynucleotides and its C-terminal do-
main, which is homologous to the ␣ and  subunits of the
F
1
–ATPase (Section 22-3Cb), binds an NTP. The X-ray
structure of Rho in complex with AMPPNP and an 8-nt
RNA, r(UC)
4
(Fig. 31-20a), determined by James Berger,
reveals that Rho forms a hexameric lock washer–shaped
helix that is 120 Å in diameter with an ⬃30-Å-diameter
central hole and whose first and sixth subunits are sepa-
rated by a 12-Å gap and a rise of 45 Å along the helix axis.
The RNAs, only a single UC unit of which is visible in each
chain, bind along the top of the helix to the so-called pri-
mary RNA binding sites on the N-terminal domains,
whereas AMPPNP binds to the C-terminal domains (which
are further from the viewer in Fig. 31-20a than the N-termi-
nal domains) at the interface between subunits. This X-ray
structure represents an open state that has bound to rut site
mRNA and is poised to bind additional mRNA upon its
entry into the central cavity through the gap.
In the X-ray structure of Rho in complex with rU
12
and
the ATP mimic ADP ⴢ BeF
3
(Fig. 31-20b), also determined
by Berger, the helicase’s six subunits have formed a closed
ring in which each subunit has a different conformation.
The RNA, only 6 nt of which are visible, assumes a right-
handed helical conformation with its 5¿ end closest to the
viewer in Fig. 31-20b and binds to Rho’s N-terminal do-
mains in the helicase’s central channel, the so-called sec-
ondary RNA binding site. Protein loops that extend from
the walls of the central channel to interact with the RNA
are helically arranged like the steps of a right-handed spiral
staircase such that they track the RNA’s sugar–phosphate
backbone, much like the central loops of E1 protein (an
AAA⫹ family hexagonal helicase) tracks its centrally
bound ssDNA (Section 30-2Ca). The different conforma-
tions of Rho’s six subunits indicate that they sequentially
undergo a series of six NTP-driven conformational
changes and that these changes are allosterically coupled
so that they progress around the hexamer in a wavelike
manner. Since each of the foregoing loops maintains its
grip on the same nucleotide during this process, the heli-
case translocates along its bound RNA, in much the same
way that E1 protein translocates along its bound ssDNA.
Why, then, do Rho and E1 protein move in opposite direc-
tions? Comparison of the structures of Rho and E1 protein
Section 31-2. RNA Polymerase 1275
JWCL281_c31_1260-1337.qxd 8/11/10 9:48 PM Page 1275