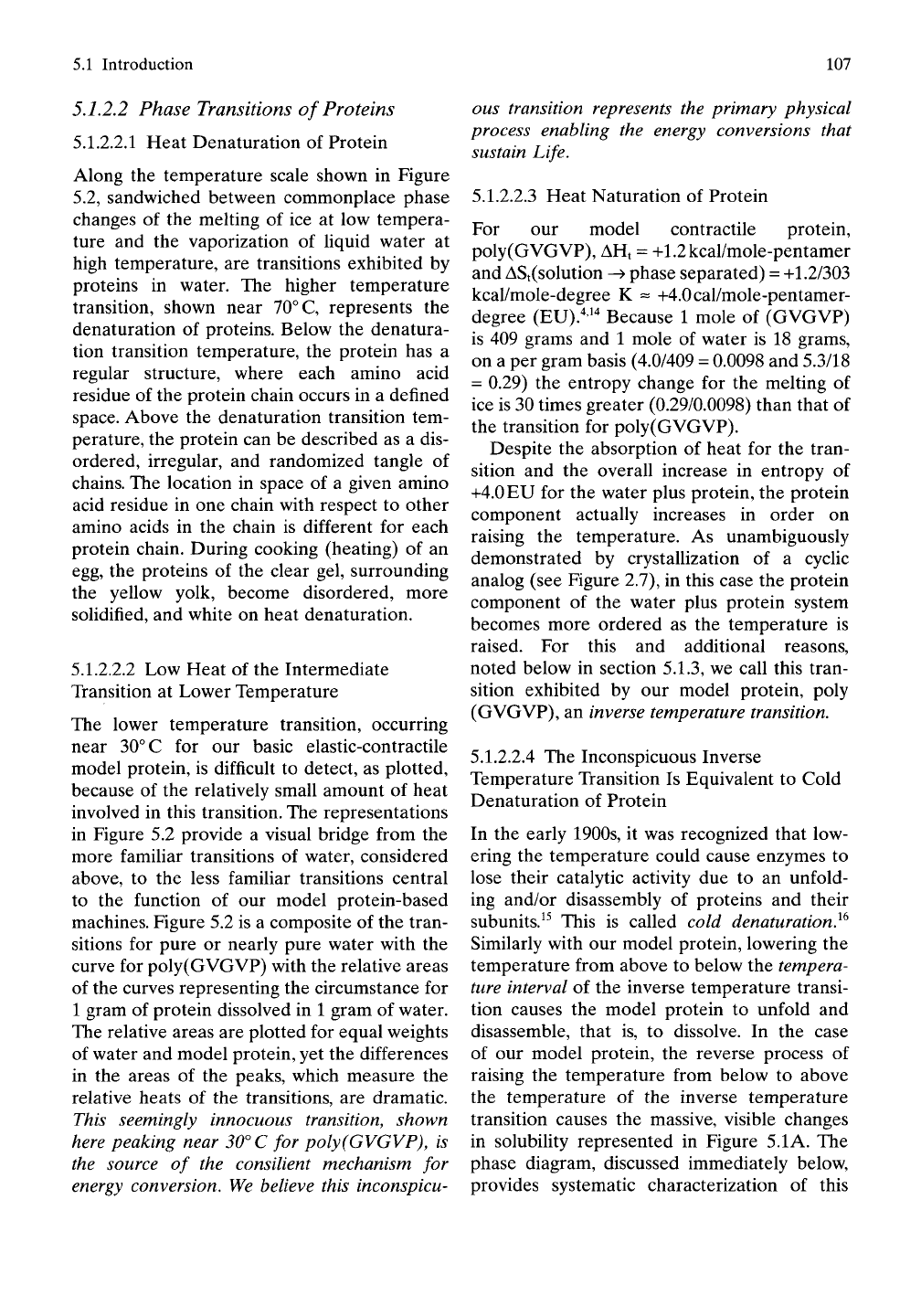
5.1 Introduction 107
5.1.2.2 Phase Transitions of Proteins
5.1.2.2.1 Heat Denaturation of Protein
Along the temperature scale shown in Figure
5.2, sandwiched between commonplace phase
changes of the melting of ice at low tempera-
ture and the vaporization of liquid water at
high temperature, are transitions exhibited by
proteins in water. The higher temperature
transition, shown near 70° C, represents the
denaturation of proteins. Below the denatura-
tion transition temperature, the protein has a
regular structure, where each amino acid
residue of the protein chain occurs in a defined
space. Above the denaturation transition tem-
perature, the protein can be described as a dis-
ordered, irregular, and randomized tangle of
chains. The location in space of a given amino
acid residue in one chain with respect to other
amino acids in the chain is different for each
protein chain. During cooking (heating) of an
egg, the proteins of the clear gel, surrounding
the yellow yolk, become disordered, more
sohdified, and white on heat denaturation.
5.1.2.2.2 Low Heat of the Intermediate
Transition at Lower Temperature
The lower temperature transition, occurring
near 30° C for our basic elastic-contractile
model protein, is difficult to detect, as plotted,
because of the relatively small amount of heat
involved in this transition. The representations
in Figure 5.2 provide a visual bridge from the
more familiar transitions of water, considered
above, to the less familiar transitions central
to the function of our model protein-based
machines. Figure 5.2 is a composite of the tran-
sitions for pure or nearly pure water with the
curve for poly(GVGVP) with the relative areas
of the curves representing the circumstance for
1 gram of protein dissolved in 1 gram of water.
The relative areas are plotted for equal weights
of water and model protein, yet the differences
in the areas of the peaks, which measure the
relative heats of the transitions, are dramatic.
This seemingly innocuous transition, shown
here peaking near
30"^
C for poly(GVGVP), is
the source of the consilient mechanism for
energy conversion. We believe this inconspicu-
ous transition represents the primary physical
process enabling the energy conversions that
sustain Life.
5.1.2.2.3 Heat Maturation of Protein
For our model contractile protein,
poly(GVGVP), AHt = -hl.2kcal/mole-pentamer
and ASt(solution -^ phase separated) = +1.2/303
kcal/mole-degree K ~ +4.0cal/mole-pentamer-
degree (EU).'''' Because 1 mole of (GVGVP)
is 409 grams and 1 mole of water is 18 grams,
on a per gram basis (4.0/409 = 0.0098 and
5.3/18
= 0.29) the entropy change for the melting of
ice is 30 times greater (0.29/0.0098) than that of
the transition for poly (GVGVP).
Despite the absorption of heat for the tran-
sition and the overall increase in entropy of
+4.0
EU for the water plus protein, the protein
component actually increases in order on
raising the temperature. As unambiguously
demonstrated by crystallization of a cyclic
analog (see Figure 2.7), in this case the protein
component of the water plus protein system
becomes more ordered as the temperature is
raised. For this and additional reasons,
noted below in section 5.1.3, we call this tran-
sition exhibited by our model protein, poly
(GVGVP), an inverse temperature transition.
5.1.2.2.4 The Inconspicuous Inverse
Temperature Transition Is Equivalent to Cold
Denaturation of Protein
In the early 1900s, it was recognized that low-
ering the temperature could cause enzymes to
lose their catalytic activity due to an unfold-
ing and/or disassembly of proteins and their
subunits.^^ This is called cold denaturation.^^
Similarly with our model protein, lowering the
temperature from above to below the tempera-
ture interval of the inverse temperature transi-
tion causes the model protein to unfold and
disassemble, that is, to dissolve. In the case
of our model protein, the reverse process of
raising the temperature from below to above
the temperature of the inverse temperature
transition causes the massive, visible changes
in solubility represented in Figure 5.1A. The
phase diagram, discussed immediately below,
provides systematic characterization of this