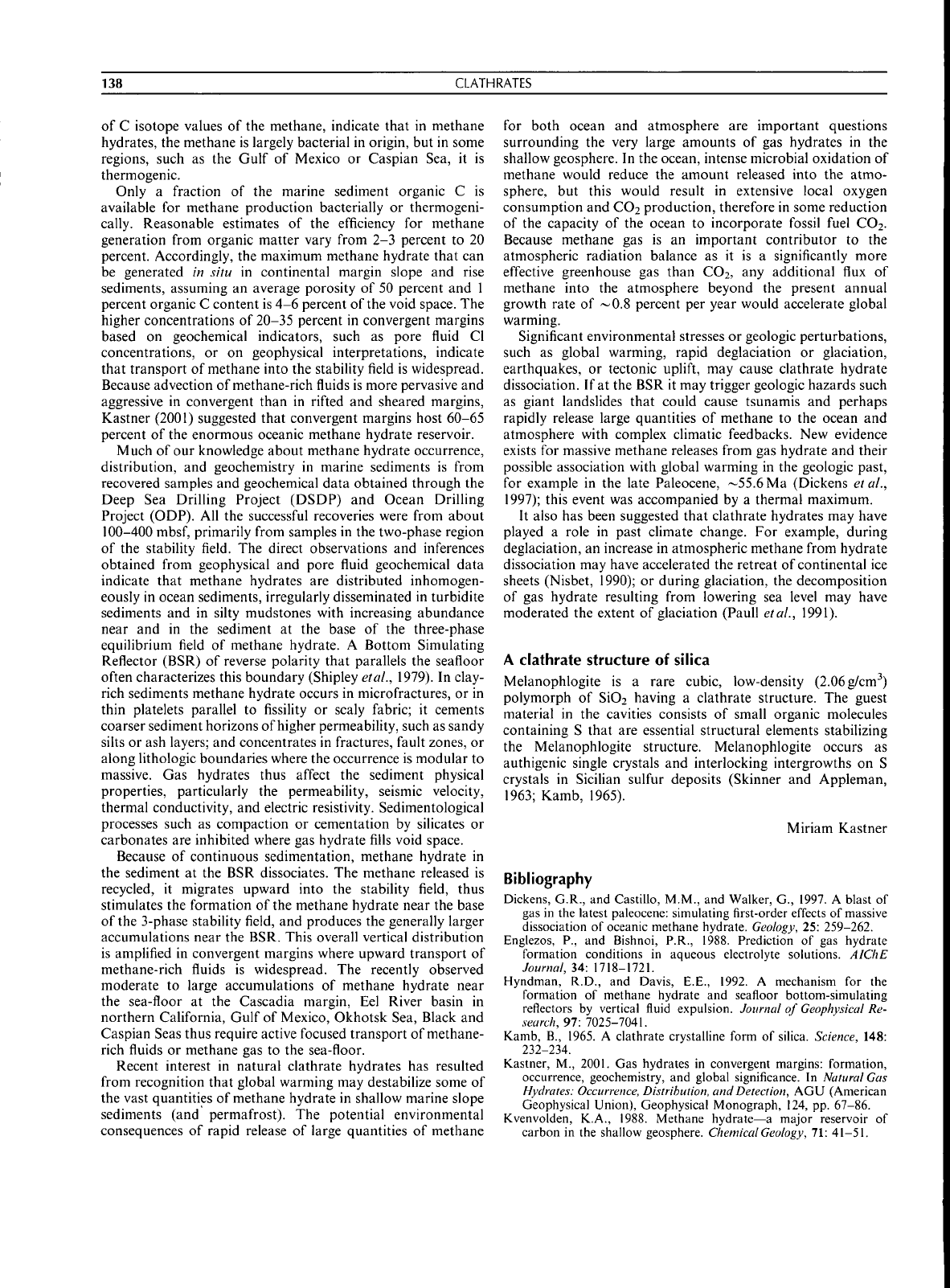
138 CLATHRATES
of C isotope values of the methane, indicate that in methane
hydrates, the methane is largely bacterial in origin, but in some
regions, such as the Gulf of Mexico or Caspian Sea, it is
thermogenic.
Only a fraction of the marine sediment organic C is
available for methane production bacterially or thermogeni-
cally. Reasonable estimates of the efficiency for methane
generation from organic matter vary from 2-3 percent to 20
percent. Accordingly, the maximum methane hydrate that can
be generated in situ in continental margin slope and rise
sediments, assuming an average porosity of 50 percent and 1
percent organic C content is 4-6 percent ofthe void space. The
higher concentrations of 20-35 percent in convergent margins
based on geochemical indicators, such as pore fluid Cl
concentrations, or on geophysical interpretations, indicate
that transport of methane into the stability field is widespread.
Because advection of methane-rich fluids is more pervasive and
aggressive in convergent than in rifted and sheared margins,
Kastner (2001) suggested that convergent margins host 60-65
percent of the enormous oceanic methane hydrate reservoir.
Much of our knowledge about methane hydrate occurrence,
distribution, and geochemistry in marine sediments is from
recovered samples and geochemical data obtained through the
Deep Sea Drilling Project (DSDP) and Ocean Drilling
Project (ODP). All the successful recoveries were from about
100-400
mbsf,
primarily from samples in the two-phase region
of the stability field. The direct observations and inferences
obtained from geophysical and pore fiuid geochemical data
indicate that methane hydrates are distributed inhomogen-
eously in ocean sediments, irregularly disseminated in turbidite
sediments and in silty mudstones with increasing abundance
near and in the sediment at the base of the three-phase
equilibrium field of methane hydrate. A Bottom Simulating
Refiector (BSR) of reverse polarity that parallels the seafioor
often characterizes this boundary (Shipley etal., 1979). In clay-
rich sediments methane hydrate occurs in mierofractures, or in
thin platelets parallel to fissility or scaly fabric; it cements
coarser sediment horizons of higher permeability, such as sandy
silts or ash layers; and concentrates in fractures, fault zones, or
along lithologic boundaries where the occurrence is modular to
massive. Gas hydrates thus affect the sediment physical
properties, particularly the permeability, seismic velocity,
thermal conductivity, and electric resistivity. Sedimentological
processes such as compaction or cementation by silicates or
carbonates are inhibited where gas hydrate fills void space.
Because of continuous sedimentation, methane hydrate in
the sediment at the BSR dissociates. The methane released is
recycled, it migrates upward into the stability field, thus
stimulates the formation of the methane hydrate near the base
of the 3-phase stability field, and produces the generally larger
accumulations near the BSR. This overall vertical distribution
is amplified in convergent margins where upward transport of
methane-rich fiuids is widespread. The recently observed
moderate to large accumulations of methane hydrate near
the sea-fioor at the Caseadia margin. Eel River basin in
northern California, Gulf of Mexico, Okhotsk Sea, Black and
Caspian Seas thus require active focused transport of methane-
rich fiuids or methane gas to the sea-fioor.
Recent interest in natural clathrate hydrates has resulted
from recognition that global warming may destabilize some of
the vast quantities of methane hydrate in shallow marine slope
sediments (and permafrost). The potential environmental
consequences of rapid release of large quantities of methane
for both ocean and atmosphere are important questions
surrounding the very large amounts of gas hydrates in the
shallow geosphere. In the ocean, intense microbial oxidation of
methane would reduce the amount released into the atmo-
sphere, but this would result in extensive local oxygen
consumption and CO2 production, therefore in some reduction
of the capacity of the ocean to incorporate fossil fuel CO2.
Because methane gas is an important contributor to the
atmospheric radiation balance as it is a significantly more
effective greenhouse gas than CO2, any additional flux of
methane into the atmosphere beyond the present annual
growth rate of ~0.8 percent per year would accelerate global
warming.
Significant environmental stresses or geologic perturbations,
such as global warming, rapid deglaciation or glaeiation,
earthquakes, or tectonic uplift, may cause clathrate hydrate
dissociation. If at the BSR it may trigger geologic hazards such
as giant landslides that could cause tsunamis and perhaps
rapidly release large quantities of methane to the ocean and
atmosphere with complex climatic feedbacks. New evidence
exists for massive methane releases from gas hydrate and their
possible association with global warming in the geologic past,
for example in the late Paleocene, ~55.6 Ma (Dickens et al.,
1997);
this event was accompanied by a thermal maximum.
It also has been suggested that clathrate hydrates may have
played a role in past climate change. For example, during
deglaciation, an increase in atmospheric methane from hydrate
dissociation may have accelerated the retreat of continental ice
sheets (Nisbet, 1990); or during glaeiation, the decomposition
of gas hydrate resulting from lowering sea level may have
moderated the extent of glaeiation (Paull etal., 1991).
A clathrate structure of silica
Melanophlogite is a rare cubic, low-density (2.06 g/cm^)
polymorph of SiO2 having a clathrate structure. The guest
material in the cavities consists of small organic molecules
containing S that are essential structural elements stabilizing
the Melanophlogite structure. Melanophlogite occurs as
authigenic single crystals and interlocking intergrowths on S
crystals in Sicilian sulfur deposits (Skinner and Appleman,
1963;
Kamb, 1965).
Miriam Kastner
Bibliography
Dickens, G.R., and Castillo, M.M., and Walker, G., 1997. A blast of
gas in the latest paleocene: simulating first-order effects of massive
dissociation of oceanic methane hydrate. Geology, 25: 259-262.
Englezos, P., and Bishnoi, P.R., 1988. Prediction of gas hydrate
formation conditions in aqueous electrolyte solutions. AIChE
Journal, 34: 1718-1721.
Hyndman, R.D., and Davis, E.E., 1992. A mechanism for the
formation of methane hydrate and seafloor bottom-simulating
reflectors by vertical fluid expulsion. Journal of Geophysical Re-
.search,
97: 7025-7041.
Kamb, B., 1965. A clathrate crystalline form of silica. Science, 148:
232-234.
Kastner, M., 2001. Gas hydrates in convergent margins: formation,
occurrence, geochemistry, and global signiflcance. In Natural Gas
Hydrates:
Occurrence,
Distribution, and Detection, AGU (American
Geophysical Union), Geophysical Monograph, 124, pp. 67-86.
Kvenvolden, K.A., 1988. Methane hydrate—a major reservoir of
carbon in the shallow geosphere. Chemical
Geology,
71:
41-51.