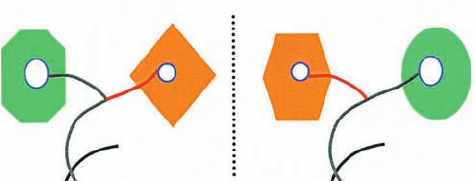
Class I
Class II
Catalytic
domain
Catalytic
domain
Editing
domain
Editing
domain
tRNA acceptor stem
tRNA acceptor stem
ThrRS, a Class II homodimer, has the opposite problem
of ValRS: It must synthesize Thr–tRNA
Thr
but not Val–
tRNA
Thr
.The X-ray structure of E. coli ThrRS that lacks its
N-terminal domain but remains catalytically active in a
complex with either threonine or the threonyl–adenylate
analog Thr-AMS, determined by Moras, reveals that
ThrRS’s aminoacylation pocket contains a Zn
2
ion that is
coordinated by the side chain hydroxyl and amino groups
of the threonyl group as well as by three protein side
chains. The isosteric valine could not coordinate the Zn
2
ion in this way and hence does not undergo adenylylation
by ThrRS. However, what prevents ThrRS from synthesiz-
ing Ser–tRNA
Thr
? In fact, the truncated ThrRS synthesizes
Ser–tRNA
Thr
at more than half the rate it synthesizes
Thr–tRNA
Thr
, thereby indicating that the N-terminal do-
main of wild-type ThrRS contains the enzyme’s editing site.
Mutational analysis of ThrRS has localized this editing site
to a cleft in the N-terminal domain of wild-type ThrRS,
whose X-ray structure in complex with tRNA
Thr
was also
determined by Moras. In this latter structure, the tRNA’s 3¿
end follows a regular helical path similar to that seen in the
X-ray structure of AspRS tRNA
Asp
ATP (Fig. 32-18) so
as to enter the aminoacylation site. However, if the 3¿ end
of the bound tRNA
Thr
assumed a hairpin conformation
similar to that seen in X-ray structure of tRNA
Gln
in com-
plex with the Class I enzyme GlnRS and ATP (Fig. 32-17),
its covalently linked aminoacyl group would enter the edit-
ing site. This indicates an intriguing “mirror symmetry”
(Fig. 32-23): In Class I aaRSs that mediate a double-sieve
editing mechanism, the 3¿ end of the bound cognate tRNA
assumes a hairpin conformation when it enters the aminoa-
cylation site and a helical conformation when it enters the
editing site, whereas the converse holds for Class II aaRSs.
Finally, ThrRS does not appear to mediate pretransfer ed-
iting (does not hydrolyze seryl–adenylate), and, in fact, the
ThrRS tRNA
Thr
complex lacks a channel connecting its
aminoacylation and editing sites such as is seen in the
ValRS tRNA
Va l
complex.
Synthetases that have adequate selectivity for their cor-
responding amino acid lack editing functions. Thus, for ex-
ample, the TyrRS aminoadenylylation site discriminates
between tyrosine and phenylalanine through hydrogen
bonding with the tyrosine ¬OH group. The cell’s other
amino acids, standard as well as nonstandard, have even
less resemblance to tyrosine, which rationalizes why TyrRS
lacks an editing site.
f. Gln–tRNA
Gln
May Be Formed via an
Alternative Pathway
Although it was long believed that each of the 20 stan-
dard amino acids is covalently linked to a tRNA by its corre-
sponding aaRS, it is now clear that gram-positive bacteria,
archaebacteria, cyanobacteria, mitochondria, and chloro-
plasts all lack GlnRS. Rather glutamate is linked to tRNA
Gln
by the same GluRS that synthesizes Glu–tRNA
Glu
. The re-
sulting Glu–tRNA
Gln
is then transamidated to Gln–tRNA
Gln
by the enzyme Glu–tRNA
Gln
amidotransferase (Glu-AdT)
in an ATP-requiring reaction in which glutamine is the
amide donor. Some microorganisms use a similar transami-
dation pathway for the synthesis of Asn–tRNA
Asn
from
Asp–tRNA
Asn
.
The overall reaction catalyzed by Glu-AdT occurs in
three stages (Fig. 32-24): (1) Glutamine is hydrolyzed to
glutamate and the resulting NH
3
sequestered; (2) ATP re-
acts with the Glu side chain of Glu–tRNA
Gln
to yield an ac-
tivated acylphosphate intermediate and ADP; and (3) the
acylphosphate intermediate reacts with the NH
3
to yield
Gln–tRNA
Gln
P
i
. Glu-AdT from Bacillus subtilis, which
was characterized by Dieter Söll, is a heterotrimeric pro-
tein, none of whose subunits exhibit significant sequence
similarity to GlnRS. The genes encoding these subunits,
gatA, gatB, and gatC, form a single operon whose disrup-
tion is lethal, thereby demonstrating that B. subtilis has no
alternative pathway for Gln–tRNA
Gln
production. The
GatA subunit of Glu-AdT appears to catalyze the activa-
tion of the side chain carboxyl of glutamic acid via a reac-
tion resembling that catalyzed by carbamoyl phosphate
synthetase (Section 26-2A). Nevertheless, GatA exhibits
no sequence similarity with other known glutamine amido-
transferases (members of the triad or Ntn families; Section
26-5Aa). The GatB subunit may be used to select the cor-
rect tRNA substrate. The role of the GatC subunit is un-
clear, although the observation that its presence is neces-
sary for the expression of GatA in E. coli suggests that it
participates in the modification, folding, and/or stabiliza-
tion of GatA.
Since Glu is not misincorporated into B. subtilis proteins
in place of Gln, the Glu–tRNA
Gln
product of the above
aminoacylation reaction must not be transported to the ri-
bosome in the same way as Gln–tRNA
Gln
. It is likely that
this occurs because, as has been shown in chloroplasts, EF-
Tu, the elongation factor that binds and transports most
bacterial aminoacyl–tRNAs to the ribosome in a GTP-
dependent process (Section 32-3D), does not bind
Glu–tRNA
Gln
. It is unclear why two independent routes
have evolved for the synthesis of Gln–tRNA
Gln
.
1358 Chapter 32. Translation
Figure 32-23 Schematic diagram of the aminoacylation and
editing mechanisms of Class I and Class II aaRSs emphasizing
the “mirror symmetry” of their overall mechanisms. With Class I
aaRSs (left; e.g., IleRS), the 3¿ end of the bound tRNA’s acceptor
stem assumes a hairpin conformation in the synthetic mode and
a helical conformation in the editing mode, whereas the converse
occurs with Class II aaRSs (right; e.g.,ThrRS). [Courtesy of Dino
Moras, CNRS/INSERM/ULP, Illkirch Cedex, France.]
JWCL281_c32_1338-1428.qxd 8/4/10 4:44 PM Page 1358