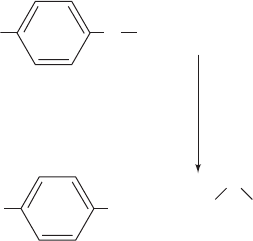
polypeptide (R1 does not crystallize satisfactorily in the
absence of this polypeptide), also determined by Eklund
(Fig. 28-12d). The central domain of the three-domain R1
monomer consists of a novel 10-stranded ␣/ barrel that is
formed by the antiparallel joining of two topologically sim-
ilar half-barrels, each comprising five parallel  strands
connected by four ␣ helices. As with the similar 8-stranded
␣/ barrels that form the active sites of numerous enzymes
(Section 8-3Bh), R1’s active site Cys residues (439, 225,
462) are located in the mouth of the 10-stranded ␣/ barrel.
The two R1 Cys residues, 754 and 759, that are impli-
cated in the regeneration of the active enzyme are compo-
nents of R1’s C-terminal segment, which is not visible in
the X-ray structure of R1 and is presumably disordered.
This observation supports the hypothesis that this C-terminal
segment acts to flexibly shuttle reducing equivalents from
the enzyme surface to its active site.
b. Radical Generation in Class I RNR Requires
the Presence of O
2
One of the most remarkable aspects of Class I RNR is
its ability to stabilize its normally highly reactive TyrOⴢ
radical (its half-life is 4 days in the protein vs milliseconds
in solution). Yet quenching the radical, say, by hydrox-
yurea, inactivates the enzyme.How, then, is the radical gen-
erated in the first place? The radical may be restored in
vitro by simply treating the inactive enzyme with Fe(II)
and a reducing agent in the presence of O
2
.
This is a four-electron reduction of O
2
in which the reduc-
ing agent that supplies the electron represented by e
–
may
be ascorbate or even excess Fe
2⫹
.
c. The Inability of Oxidized RNR to Bind Substrate
Serves an Important Protective Function
Comparison of the X-ray structures of reduced R1 (in
which the redox-active Cys 225 and Cys 462 residues are in
their SH forms) with that of oxidized R1 (in which Cys 225
and Cys 462 are disulfide linked) reveals that Cys 462 in re-
duced R1 has rotated away from its position in oxidized R1
to become buried in a hydrophobic pocket, whereas Cys 225
moves into the region formerly occupied by Cys 462.The dis-
tance between the formerly disulfide-linked S atoms thereby
increases from 2.0 to 5.7 Å.These movements are accompa-
nied by small shifts of the surrounding polypeptide chain.
Oxidized RNR does not bind substrate because its R1 Cys
OO
2
H
Tyr 122-R2
O•
++++2Fe
2+
+ Fe
3+
+ H
2
OFe
3+
O
2
_
H
+
e
Tyr 122-R2
225 would prevent the binding of substrate through steric in-
terference of its S atom with the substrate NDP’s O2¿ atom.
The inability of oxidized RNR to bind substrate has
functional significance. In the absence of substrate, the en-
zyme’s free radical is stored in the interior of the R2 pro-
tein, close to its dinuclear iron center. When substrate is
bound, the radical is presumably transferred to it via a se-
ries of protein side chains in both R2 and R1. If the sub-
strate is unable to properly react after accepting this free
radical, as would be the case if RNR were in its oxidized
state, this could result in the destruction of the substrate
and/or the enzyme. Indeed, the mutation of the redox-
active Cys 225 to Ser results in an enzyme that permits the
formation of the substrate radical (Fig. 28-13); however,
since the mutant enzyme is incapable of reducing it,the sub-
strate radical instead decomposes followed by the release of
its base and phosphate moieties. More importantly, a tran-
sient peptide radical forms, which cleaves and inactivates
the R1 polypeptide chain while consuming the radical and
thereby inactivating R2. Thus, an important role of the en-
zyme is to control the release of the radical’s powerful oxi-
dizing capability. It does so in part by preventing the bind-
ing of substrate while the enzyme is in its oxidized form.
d. Ribonucleotide Reductase Is Regulated by
Effector-Induced Oligomerization
The synthesis of the four dNTPs in the amounts re-
quired for DNA synthesis is accomplished through feed-
back control. The maintenance of the proper intracellular
ratios of dNTPs is essential for normal growth. Indeed, a
deficiency of any dNTP is lethal, whereas an excess is muta-
genic because the probability that a given dNTP will be
erroneously incorporated into a growing DNA strand
increases with its concentration relative to those of the other
dNTPs.
The activities of both E. coli and mammalian Class I
RNRs are allosterically responsive to the concentrations of
various (d)NTPs. Thus, as Reichard has shown, ATP in-
duces the reduction of CDP and UDP; dTTP induces the
reduction of GDP and inhibits the reduction of CDP and
UDP; dGTP induces the reduction of ADP and, in mam-
mals but not E. coli, inhibits the reduction of CDP and
UDP; and dATP inhibits the reduction of all NDPs.
Barry Cooperman has shown that the catalytic activity
of mouse RNR varies with its state of oligomerization (that
is, RNR is a morpheein; Section 26-4Ac), which in turn is
governed by the binding of nucleotide effectors to three in-
dependent allosteric sites on R1: (1) the specificity site,
which binds ATP, dATP, dGTP, and dTTP; (2) the activity
site, which binds ATP and dATP; and (3) the hexameriza-
tion site, which binds only ATP. On the basis of molecular
mass, ligand binding, and activity studies on mouse RNR,
Cooperman formulated a model that quantitatively ac-
counts for the allosteric regulation of Class I RNR. It has
the following features (Fig. 28-14a):
1. The binding of ATP, dATP, dGTP, or dTTP to the
specificity site induces the catalytically inactive R1
monomers to form a catalytically active dimer, R1
2
.
1122 Chapter 28. Nucleotide Metabolism
JWCL281_c28_1107-1142.qxd 8/9/10 9:46 AM Page 1122