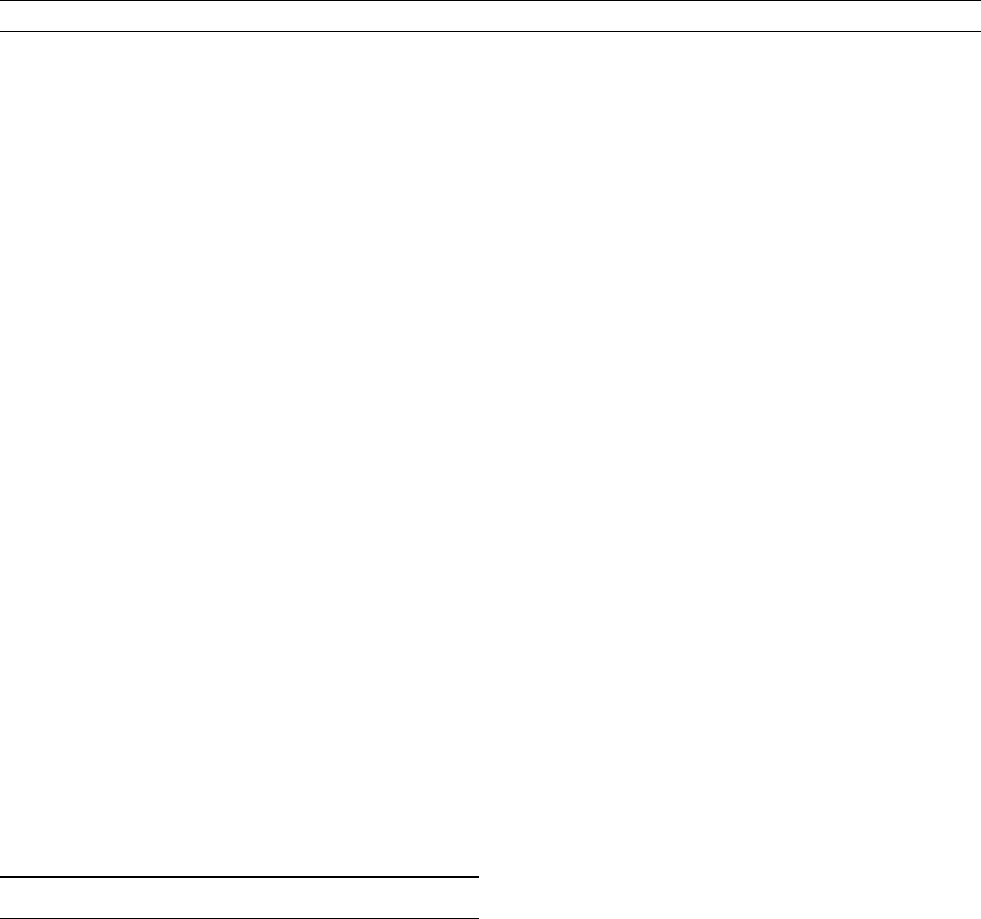
McNeill, J.D., 1980. Electromagnetic terrain conductivity measure-
ment at low induction numbers. Technical Note TN-6, Geonics
Limited, Mississauga, Ontario.
McNeill, J.D., and Labson, V., 1991. Geological mapping using VLF
radio fields. In Nabighian, M.N. (ed.), Electromagnetic Methods
in Applied Geophysics. Tulsa, OK: Society of Exploration Geophy-
sicists, pp. 521–640.
McNeill, J.D., 1990. Use of electromagnetic methods for groundwater
studies. In Ward, S.H. (ed.), Geotechnical and Environmental
Geophysics. Tulsa, OK: Society of Exploration Geophysicists,
pp. 191–218.
Nabighian, M.N., and Macnae, J.C., 1991. Time domain electromag-
netic prospecting methods. In Nabighian, M.N. (ed.), Electromag-
netic Methods in Applied Geophysics. Tulsa, OK: Society of
Exploration Geophysicists, pp. 427–520.
Olhoeft, G.R., 1985. Low-frequency electrical properties. Geophysics,
50: 2492–2503.
Palacky, G.J., and West, G.F., 1991. Airborne electromagnetic meth-
ods. In Nabighian, M.N. (ed.), Electromagnetic Methods in Applied
Geophysics. Tulsa, OK: Society of Exploration Geophysicists,
pp. 811– 880.
Vozoff, K., 1991. The magnetotelluric method. In Nabighian, M.N.
(ed.), Electromagnetic Methods in Applied Geophysics. Tulsa,
OK: Society of Exploration Geophysicists, pp. 641–712.
Zonge, K.L., and Hughes L.J., 1991. Controlled source audio-
frequency magnetotellurics. In Nabighian, M.N. (ed.), Electro-
magnetic Methods in Applied Geophysics. Tulsa, OK: Society of
Exploration Geophysicists, pp. 713–810.
Cross-references
EM Modeling, Forward
EM Modeling, Inverse
EM, Industrial Uses
EM, Land Uses
EM, Marine Controlled Source
Magnetometers, Laboratory
Magnetotellurics
Transient EM Induction
EM, LAKE-BOTTOM MEASUREMENTS
Magnetic fields set up by electrical currents flowing in the Earth's
ionosphere and magnetosphere diffuse into the Earth's interior, and
induce electrical currents to flow in the oceans and in conductive
regions in the Earth's interior. Longer period magnetic field variations
have a greater depth of penetration than shorter period variations. By
relating the variations in the electric field to those in the magnetic field
at different locations and over a range of different periods, it is possi-
ble to reconstruct the distribution of electrical conductivity within the
Earth, and from that information, to constrain the temperature, compo-
sition, fluid and volatile content, and state of Earth materials. This
is the magnetotelluric (MT) method (see Magnetotellurics; Galvanic
distortion; EM, land uses).
In order to penetrate deep into the lithosphere and below, long-
period MT measurements are required. Other methods involving only
magnetic field measurements (see Geomagnetic deep sounding; EM,
regional studies) are valid for field variations with periods longer than
2–4 days, and provide information on electrical conductivity of the
mantle at depths below 400 km (Schultz et al ., 1987) (see Mantle,
electrical conductivity, mineralogy). Conversely, the MT method is
usually employed to investigate periods many decades shorter than
this, generally less than 0.2 days. Consequently most MT investiga-
tions are restricted to the crust and uppermost mantle. By extending
the range of MT investigations to longer periods, finer structural
details may be resolved at depth, and information on anisotropy within
the crust and mantle may be returned.
The electric field is measured by monitoring the electrical potential
across (typically) two orthogonal pairs of metal salt electrodes grounded
to Earth and separated by a fixed distance. Electrode noise is a considera-
tion for MT. There is long-period electric field measurement drift due to
variations in electrochemical conditions in the soil, moisture content, and
thermal effects (Perrier and Morat, 2000), and to electrokinetic effects (Tri-
que et al., 2002). To improve the signal-to-noise ratio, a number of deeply
penetrating MT investigations have taken place making use of extremely
long electrode separations, by using leased telephone cables on land
(Egbert et al., 1992), or abandoned submarine telecommunications cables
on the seafloor (see Conductivity, ocean floor measurements).
Schultz and Larsen (1987) proposed that the thermally and electro-
chemically stable environment of lake bottoms might provide an ideal
environment to extend MT into periods of 0.2–4 days and below, thus
spanning the gap in coverage left by conventional MT and magnetic
field methods. While lake-bottom MT shares common features with
ocean bottom MT, the high conductance of marine sediments and the
conductive seawater overburden at most marine study sites shields
the seafloor from the high frequency signals that penetrate to the bot-
tom of lakes, and that can provide higher resolution information on
shallow crustal and upper mantle conductivity structure. While con-
tamination of the EM fields by water motions (such as mesoscale
eddies) impose a low frequency limit to MT in the oceans, this is gen-
erally not a significant consideration in lakes.
A prototype lake-bottom MT station was installed in Lake Washing-
ton, in Seattle, Washington, to form an array of Ag-AgCl electrodes,
with electrode separations of 800–1300 m. This configuration pro-
vided three nonorthogonal components of the electric field, any
two of which could be rotated into orthogonal components. A fluxgate
magnetometer was buried on the adjacent shore, and high-quality MT
data resulted. The MT regional strike direction was seen to be coinci-
dent with the strike delineated by regional seismicity and tectonics.
The effects of a channeled current were seen, attributed to an Eocene
suture zone to the south of the site (Schultz et al., 1987).
A multiyear lake-bottom MT deployment followed in the Archean
Superior craton of northern Ontario, Canada (Schultz et al., 1987).
More than 2 years of MT data were collected from Carty Lake, follow-
ing the configuration at Lake Washington. Three zones in the mantle
were identified, centered at depths of 280, 456, and 825 km, and
roughly coincident with major seismic discontinuities, where the con-
ductivity increased abruptly (Figure E14). The ability to discriminate
the jump centered at around 456 km was attributed to MT data with
periods of 1 h to 1 week, which were made possible by the stable
lake-bottom installation. No other MT experiment to date has produced
resolving power sufficient to discriminate the 456 km conductivity
increase. The conductance of the upper mantle here was roughly an
order of magnitude higher than that predicted by a standard olivine
model using a standard geotherm. It was determined that either the
upper mantle geotherm was erroneously biased downward, or a dry
olivine upper mantle model was inappropriate.
Jones et al. (2001a,b, 2003) report on the lake-bottom deployment
of compact MT instruments designed for marine use, and of electric
dipole receivers inserted through the ice to the bottom of frozen lakes,
with magnetometers nearby on shore. These installations in roughly
20 lakes were made to probe to the depth of the top of the asthenosphere
beneath the Slave craton in northwestern Canada, in a region of
diamondiferous kimberlite pipes. Their investigations serendipitously
revealed a highly conductive zone at depths of 80 to below 100 km
in the mantle that is interpreted to represent interconnected carbon or
graphite along mineral grain faces. Jones et al. (2003, 2004) determined
that the Superior craton MT data of Schultz et al. (1993) and those of the
Slave craton indicate that while both provinces differ in shallow structure,
on average the deeper mantle sections are nearly identical. Jones et al.
(2003) compared the lake-bottom MT data against earlier data from the
region, and found that these depths had previously been beyond the
EM, LAKE-BOTTOM MEASUREMENTS 227