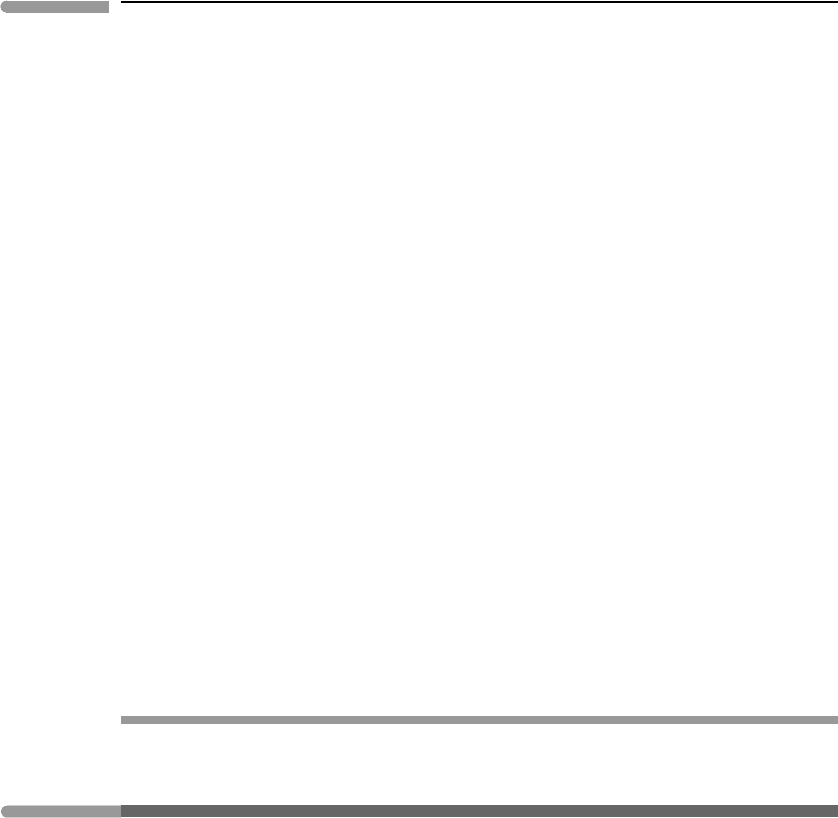
78 Energy sources in planetary bodies
we see that, with one notable exception, planetary binding energies are one to two orders
of magnitude greater than the observed energy outputs or, in other words, observed heat
fluxes are significantly less than those that could be expected from steady release of all of
the gravitational binding energy over the age of the Solar System. This discrepancy can be
the result of a combination of at least three factors: (i) early in their histories the planets
cooled at rates that were much faster than today’s, (ii) heat transfer inside the planets is
very inefficient, so that a large fraction of the dissipated binding energy is still stored inside
the planets, and (iii) only a fraction of their gravitational binding energies were retained as
internal energy, with the balance being radiated to space during planet formation.
The one notable exception to these conclusions is Io, for which measured heat flow is
two orders of magnitude higher than what can be accounted for from gravitational binding
energy. The conclusion in this case is that Io, which is the most volcanically active planetary
body in the Solar System (see, for example, Davies, 2001) must have a major heat source
that is not active to any significant extent in any of the other bodies included in Fig. 2.4.
Focusing now on the planets that appear to show an excess of gravitational binding
energy, we see that there are some subtle differences. The ratio of binding energy to heat
flow for Earth, Jupiter and Neptune is almost exactly the same (q
B
/q
M
≈ 40). Compared
to these three planets, Saturn puts out twice as much heat relative to its binding energy
(q
B
/q
M
≈18), whereas Uranus radiates one sixth less heat (q
B
/q
M
≈240). Saturn’s large
energy output is thought to reflect a process that is not significant at present in any other
planet (see Stevenson & Salpeter, 1977; Hubbard, 1980; see also Section 2.6).
Martian heat flow has not been measured yet. The value quoted in Table 2.1 and plotted in
Fig. 2.4 is an estimate based on the bulk chemical composition of Mars, which appears to be
enriched in K relative to the Earth. Lunar heat flow was measured in two of the Apollo land-
ing sites. If these values are representative of the entire Moon then, by comparison with other
terrestrial planets the Moon’s internal heat flux is greater than expected. This is rather sur-
prising, and may be a relic of a short-lived extreme heating event in the Moon’s early history.
2.4 Accretion
It is virtually certain that the chief process in the assemblage of the terrestrial planets was
accretion of smaller bodies (called planetesimals), which in turn were the products of accre-
tion of yet smaller bodies, and so on down to the smallest particles of dust and ice which
formed by condensation of gaseous elements in the solar nebula (see Weidenschilling, 1974,
1976, 1980, 2000; Wetherill, 1990, 1994).Although the details of the accretionary processes
that led from microscopic dust particles to Earth-size planets may not be fully understood
it is possible to place some constraints on its energetic aspects. The gravitational binding
energy of a planet is an upper bound to the amount of internal energy that can be derived from
dissipation of gravitational potential energy. The results of Worked Example 2.1 suggest,
however, that only a fraction of a planet’s gravitational binding energy may be converted to
internal energy. Some of the gravitational potential energy dissipated during accretion must
be radiated to space, however, and the radiated energy flux, given by the Stefan–Boltzmann
law (equation 2.1) is a very strong function of temperature, as it scales with the fourth-power
of T .
We want to find out what fraction of a planet’s gravitational binding energy is stored as
internal energy during accretion. Let us assume that a planetary body grows by accretion at