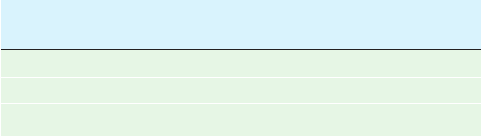
herbicides are specific inhibitors of some of these enzymes.
Such herbicides have little toxicity toward animals and hence
pose minimal risk to human health and the environment.
a. The Aspartate Family: Lysine, Methionine,
and Threonine
In bacteria, aspartate is the common precursor of lysine,
methionine, and threonine (Fig. 26-60).The biosyntheses of
these essential amino acids all begin with the aspartoki-
nase-catalyzed phosphorylation of aspartate to yield
aspartyl--phosphate. We have seen that the control of
metabolic pathways commonly occurs at the first commit-
ted step of the pathway. One might therefore expect lysine,
methionine, and threonine biosynthesis to be controlled as
a group. Each of these pathways is, in fact, independently
controlled. E. coli does so via three isozymes of aspartoki-
nase that respond differently to the three amino acids in
terms both of feedback inhibition of enzyme activity and
repression of enzyme synthesis. Table 26-3 summarizes this
differential control. In addition, the pathway direction is
controlled by feedback inhibition at the branch points by
the individual amino acids. Thus methionine inhibits the
O-acylation of homoserine (Fig. 26-60, Reaction 6), and
lysine inhibits dihydrodipicolinate synthase (Fig. 26-60,
Reaction 10).
b.
The Pyruvate Family: Leucine, Isoleucine,
and Valine
Valine and isoleucine are both synthesized via the same
five-step pathway (Fig. 26-61), the only difference being in
the first step of the series. In this TPP-dependent reaction,
which resembles those catalyzed by pyruvate decarboxy-
lase (Section 17-3Ba) and transketolase (Section 23-4Ca),
pyruvate forms an adduct with TPP, which is decarboxy-
lated to hydroxyethyl-TPP. This resonance-stabilized car-
banion adds either to the keto group of a second pyruvate
to form acetolactate on the way to valine, or to the keto
group of threonine-derived ␣-ketobutyrate to form
␣-aceto-␣-hydroxybutyrate on the way to isoleucine. The
leucine biosynthetic pathway branches off from the valine
pathway at ␣-ketoisovalerate (Fig. 26-61, Reaction 6). Re-
actions 6 to 8 in Fig. 26-61 are reminiscent of the first three
reactions of the citric acid cycle (Sections 21-3A–C). Here,
acetyl-CoA condenses with ␣-ketoisovalerate to form
␣-isopropylmalate, which then undergoes a dehydration/
hydration reaction, followed by oxidative decarboxylation
and transamination, to yield leucine.
c. The Aromatic Amino Acids: Phenylalanine,
Tyrosine, and Tryptophan
The precursors to the aromatic amino acids are the gly-
colytic intermediate phosphoenolpyruvate (PEP) and
erythrose-4-phosphate (an intermediate in the pentose
phosphate pathway; Section 23-4Cb). Their condensation
forms 2-keto-3-deoxy-
D-arabinoheptulosonate-7-phosphate,
a C
7
compound that cyclizes and is ultimately converted to
chorismate (Fig. 26-62), the branch point for tryptophan
biosynthesis. Chorismate is converted either to anthrani-
late and then on to tryptophan, or to prephenate and on to
either tyrosine or phenylalanine (Fig. 26-63). Although
mammals synthesize tyrosine by the hydroxylation of
phenylalanine (Section 26-3Ha), many microorganisms
synthesize it directly from prephenate.
Since the synthesis of aromatic amino acids only occurs
in plants and microorganisms, this pathway is a natural tar-
get for herbicides that will not be toxic to animals. For ex-
ample, glyphosate,
the active ingredient in one of the most widely used weed
killers, Roundup, is a competitive inhibitor with respect to
PEP in the 5-enolpyruvylshikimate-3-phosphate (EPSP)
synthase reaction (Reaction 6 of Fig. 26-62).
d. A Protein Tunnel Channels the Intermediate
Product of Tryptophan Synthase between
Two Active Sites
The final two reactions in tryptophan biosynthesis, Re-
actions 5 and 6 in Fig. 26-63, are both catalyzed by trypto-
phan synthase:
1. The ␣ subunit (268 residues) of this ␣
2

2
bifunctional
enzyme cleaves indole-3-glycerol phosphate, yielding in-
dole and glyceraldehyde-3-phosphate (Reaction 5).
2. The  subunit (396 residues) joins indole with
L-serine in a PLP-dependent reaction to form L-tryptophan
(Reaction 6).
Either subunit alone is enzymatically active, but when they
are joined in the ␣
2

2
tetramer, the rates of both reactions
Glyphosate
⫺2
O
3
P ¬ CH
2
¬ NH ¬ CH
2
¬ COO
⫺
Section 26-5. Amino Acid Biosynthesis 1075
Table 26-3 Differential Control of Aspartokinase
Isoenzymes in E. Coli
Feedback
Enzyme Inhibitor Corepressor(s)
a
Aspartokinase I Threonine Threonine and isoleucine
Aspartokinase II None Methionine
Aspartokinase III Lysine Lysine
a
Compounds whose presence results in the transcriptional repression of
enzyme synthesis (Section 31-3G).
Figure 26-61 (Opposite) The biosynthesis of the “pyruvate
family” of amino acids: isoleucine, leucine, and valine. The
pathway enzymes are (1) acetolactate synthase (a TPP enzyme),
(2) acetolactate mutase, (3) reductase, (4) dihydroxy acid
dehydratase, (5) valine aminotransferase (a PLP enzyme),
(6) ␣-isopropylmalate synthase, (7) ␣-isopropylmalate
dehydratase, (8) isopropylmalate dehydrogenase, (9) leucine
aminotransferase (a PLP enzyme), and (10) threonine deaminase
(serine dehydratase, a PLP enzyme).
JWCL281_c26_1019-1087.qxd 6/8/10 9:38 AM Page 1075