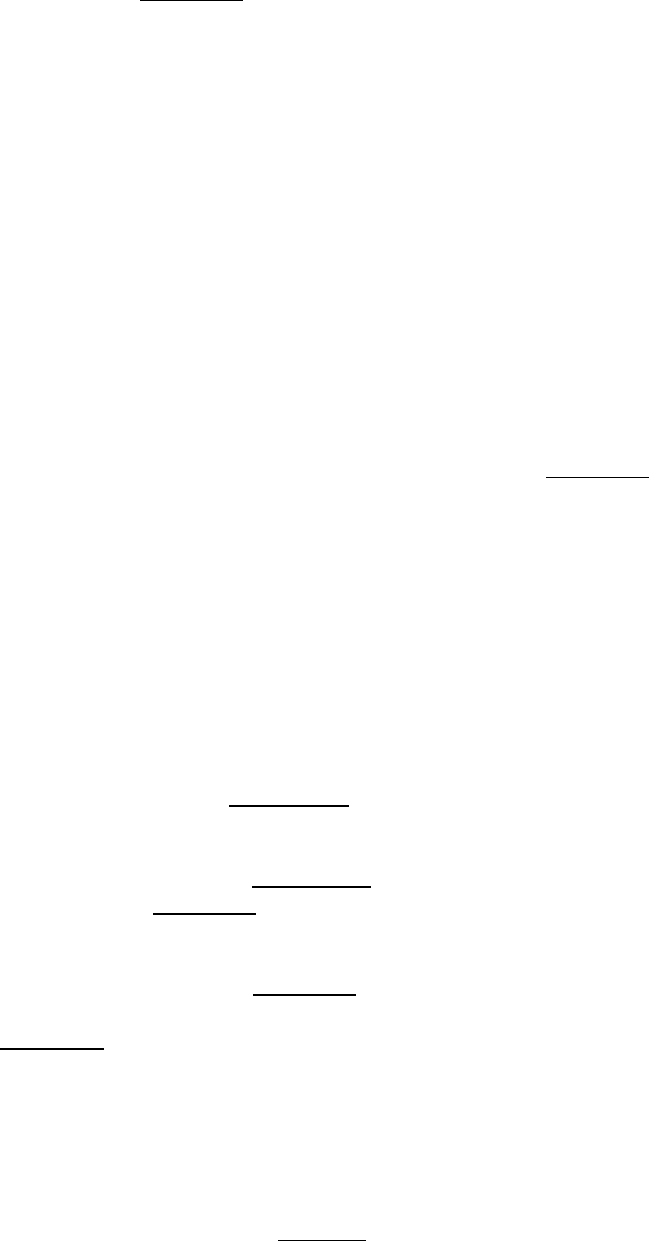
field is applied (Figure 4.43). The energy difference between these states is proportional to the strength of the imposed
magnetic field. The α state has a slightly lower energy and hence is slightly more populated (by a factor of the order of
1.00001 in a typical experiment) because it is aligned with the field. A spinning proton in an α state can be raised to an
excited state ( β state) by applying a pulse of electromagnetic radiation (a radio-frequency, or RF, pulse), provided the
frequency corresponds to the energy difference between the α and the β states. In these circumstances, the spin will
change from α to β ; in other words, resonance will be obtained. A resonance spectrum for a molecule can be obtained
by varying the magnetic field at a constant frequency of electromagnetic radiation or by keeping the magnetic field
constant and varying electromagnetic radiation.
These properties can be used to examine the chemical surroundings of the hydrogen nucleus. The flow of electrons
around a magnetic nucleus generates a small local magnetic field that opposes the applied field. The degree of such
shielding depends on the surrounding electron density. Consequently, nuclei in different environments will change states,
or resonate, at slightly different field strengths or radiation frequencies. The nuclei of the perturbed sample absorb
electromagnetic radiation at a frequency that can be measured. The different frequencies, termed chemical shifts, are
expressed in fractional units δ (parts per million, or ppm) relative to the shifts of a standard compound, such as a water-
soluble derivative of tetramethysilane, that is added with the sample. For example, a -CH
3
proton typically exhibits a
chemical shift ( δ ) of 1 ppm, compared with a chemical shift of 7 ppm for an aromatic proton. The chemical shifts of
most protons in protein molecules fall between 0 and 9 ppm (Figure 4.44). It is possible to resolve most protons in many
proteins by using this technique of onedimensional NMR. With this information, we can then deduce changes to a
particular chemical group under different conditions, such as the conformational change of a protein from a disordered
structure to an α helix in response to a change in pH.
We can garner even more information by examining how the spins on different protons affect their neighbors. By
inducing a transient magnetization in a sample through the application a radio-frequency pulse, it is possible to alter the
spin on one nucleus and examine the effect on the spin of a neighboring nucleus. Especially revealing is a two-
dimensional spectrum obtained by nuclear Overhauser enhancement spectroscopy (NOESY), which graphically displays
pairs of protons that are in close proximity, even if they are not close together in the primary structure. The basis for this
technique is the nuclear Overhauser effect (NOE), an interaction between nuclei that is proportional to the inverse sixth
power of the distance between them. Magnetization is transferred from an excited nucleus to an unexcited one if they are
less than about 5 Å apart (Figure 4.45A). In other words, the effect provides a means of detecting the location of atoms
relative to one another in the three-dimensional structure of the protein. The diagonal of a NOESY spectrum corresponds
to a one-dimensional spectrum. The offdiagonal peaks provide crucial new information: they identify pairs of protons
that are less than 5 Å apart (Figure 4.45B). A two-dimensional NOESY spectrum for a protein comprising 55 amino
acids is shown in Figure 4.46. The large number of off-diagonal peaks reveals short proton-proton distances. The three-
dimensional structure of a protein can be reconstructed with the use of such proximity relations. Structures are calculated
such that protons that must be separated by less than 5 Å on the basis of NOESY spectra are close to one another in the
three-dimensional structure (Figure 4.47). If a sufficient number of distance constraints are applied, the three-
dimensional structure can be determined nearly uniquely. A family of related structures is generated for three reasons
(Figure 4.48). First, not enough constraints may be experimentally accessible to fully specify the structure. Second, the
distances obtained from analysis of the NOESY spectrum are only approximate. Finally, the experimental observations
are made not on single molecules but on a large number of molecules in solution that may have slightly different
structures at any given moment. Thus, the family of structures generated from NMR structure analysis indicates the
range of conformations for the protein in solution. At present, NMR spectroscopy can determine the structures of only
relatively small proteins (<40 kd), but its resolving power is certain to increase. The power of NMR has been greatly
enhanced by the ability to produce proteins labeled uniformly or at specific sites with
13
C,
15
N, and
2
H with the use of
recombinant DNA technology (Chapter 6).
4.5.2. X-Ray Crystallography Reveals Three-Dimensional Structure in Atomic Detail
X-ray crystallography provides the finest visualization of protein structure currently available. This technique can reveal
the precise three-dimensional positions of most atoms in a protein molecule. The use of x-rays provides the best
resolution because the wavelength of x-rays is about the same length as that of a covalent bond. The three components in