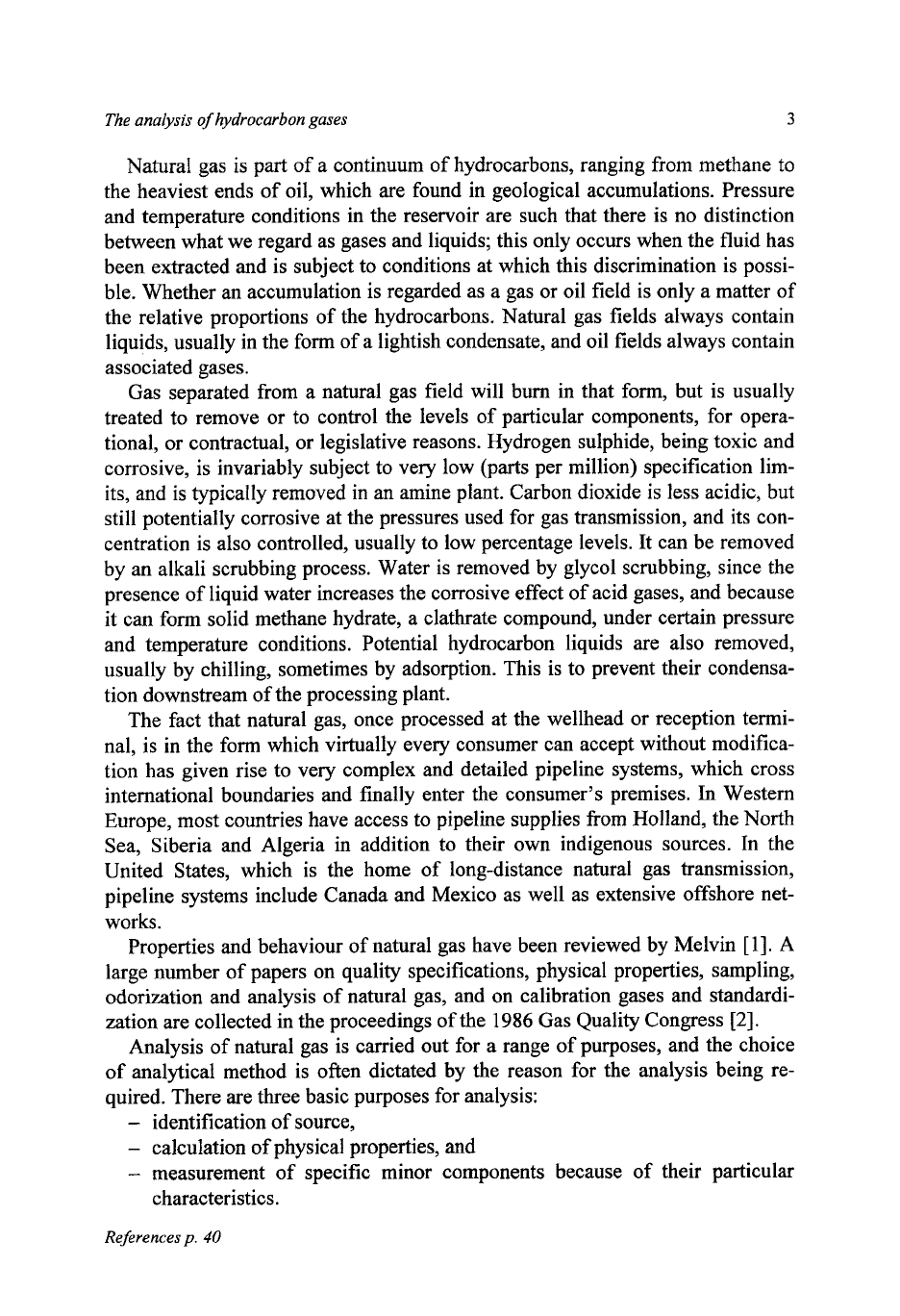
The analysis
of
hydrocarbon gases
3
Natural gas is part of a continuum of hydrocarbons, ranging from methane to
the heaviest ends of oil, which are found in geological accumulations. Pressure
and temperature conditions in the reservoir are such that there is no distinction
between what we regard as gases and liquids; this only occurs when the fluid has
been extracted and is subject to conditions at which this discrimination is possi-
ble. Whether an accumulation is regarded as a gas or oil field is only a matter of
the relative proportions of the hydrocarbons. Natural gas fields always contain
liquids, usually in the form of a lightish condensate, and oil fields always contain
associated gases.
Gas separated from a natural gas field will burn in that form, but is usually
treated to remove or to control the levels of particular components, for opera-
tional, or contractual, or legislative reasons. Hydrogen sulphide, being toxic and
corrosive, is invariably subject to very low
(parts
per million) specification lim-
its,
and is typically removed in an amine plant. Carbon dioxide is
less
acidic,
but
still potentially corrosive at the pressures used for gas transmission, and its con-
centration is also controlled, usually to low percentage levels. It can be removed
by an alkali scrubbing process. Water is removed by glycol scrubbing, since the
presence of liquid water increases the corrosive effect of acid gases, and because
it can form solid methane hydrate, a clathrate compound, under certain pressure
and temperature conditions. Potential hydrocarbon liquids are also removed,
usually by chilling, sometimes by adsorption. This is to prevent their condensa-
tion downstream of the processing plant.
The fact that natural gas, once processed at the wellhead
or
reception termi-
nal, is in the form which virtually every consumer can accept without modifica-
tion has given rise to very complex and detailed pipeline systems, which cross
international boundaries and finally enter the consumer’s premises.
In
Western
Europe,
most
countries have access to pipeline supplies from Holland, the North
Sea, Siberia and Algeria in addition to their own indigenous sources. In the
United States, which is the home of long-distance natural gas transmission,
pipeline systems include Canada and Mexico as well as extensive offshore net-
works.
Properties and behaviour of natural gas have been reviewed by Melvin
[
13.
A
large number of papers on quality specifications, physical properties, sampling,
odorization and analysis of natural gas, and on calibration gases and standardi-
zation are collected in the proceedings of the
1986
Gas Quality Congress [2].
Analysis of natural gas is carried out for a range of purposes, and the choice
of analytical method is often dictated by the reason for the analysis being re-
quired. There are three basic purposes for analysis:
-
identification of source,
-
calculation of physical properties, and
-
measurement of specific minor components because of their particular
characteristics.
References
p.
40