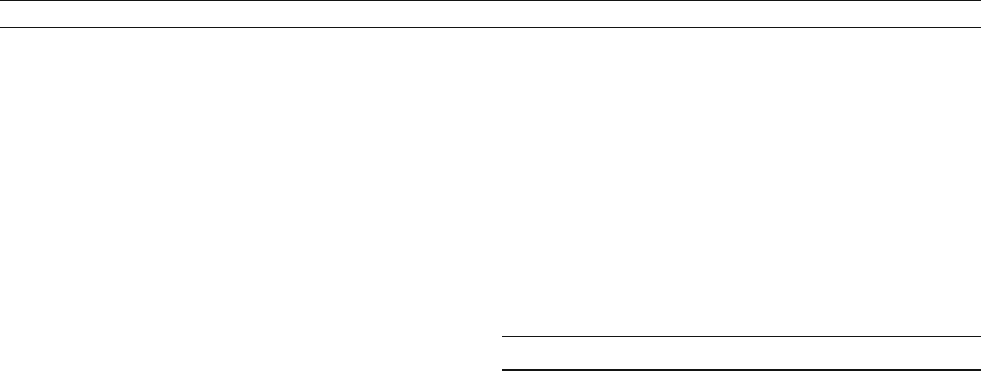
compositions and as a consequence, different refractive indices
determining their optical properties. For example, Saharan dust
is richer in iron and therefore darker and redder than Asian
dust. Refractive indices for different minerals span a wide
range for solar and thermal wavelengths (Sokolik and Toon,
1999). Data on dust refractive indices are currently limited to
only a few regions.
Dust effects on climate
Dust can influence the climate in several ways. Aerosols signif-
icantly impact on the climate system by changing the energy
balance of solar and thermal radiation (Houghton et al.,
2001). Dust not only scatters but also partly absorbs incoming
solar radiation, and also absorbs and emits outgoing long-wave
radiation. Any changes in atmospheric dust loads, like the
increase in dust during glacial periods, would cause a change
in the radiation balance and consequentially, in surface tem-
peratures. The magnitude and even the sign of the direct radia-
tive dust forcing is uncertain; it depends on the optical
properties of the dust, cloud cover, and the albedo of the under-
lying surface (Liao and Seinfeld, 1998).
The presence of dust may alter cloud optical properties by
changing the number of cloud condensation nuclei. The effi-
ciency of dust particles in forming nuclei for water clouds may
alter during transport due to mixing with soluble aerosol species.
This can influence both the brightness of clouds and the forma-
tion of rainfall. Dust particles can potentially change chemical
reactions in the atmosphere because of their large surface areas,
and therefore have a significant impact on the ozone and nitro-
gen cycles. Micronutrients (e.g., Fe) deposited with dust aerosol
possibly impact the productivity of marine and terrestrial ecosys-
tems, thus influencing the carbon cycle and potentially changing
the atmospheric greenhouse gas content. The impacts of these
indirect dust effects on climate are uncertain, since the underly-
ing processes are not yet fully understood.
Ina Tegen
Bibliography
Duce, R.A., 1995. Sources, distributions, and fluxes of mineral aerosols and
their relationship to climate. In Charlson, R.J., and Heintzenberg, J.
(eds.), Aerosol Forcing of Climate: Report of the Dahlem Workshop on
Aerosol Forcing of Climate, Berlin 1994, April 24–29, Chichester:
Wiley. pp. 43–72.
Gillette, D.A., and Passi, R., 1988. Modeling dust emission caused by wind
erosion. J. Geophys. Res., 93, 14,233–14,242.
Ginoux, P., Chin, M., Tegen, I., Prospero, J.M., Holben, B., Dubovik, O.,
and Lin, S.-J., 2001. Sources and distributions of dust aerosols simu-
lated with the GOCART model. J. Geophys. Res., 106, 20255–20273.
Houghton, J.T., Ding, Y., Griggs, D.J., Noguer, M., van der Linden, P.J.,
Dai, X., Maskell, K., and Johnon, C.A. (eds.), 2001. Climate Change.
New York, NY: Cambridge University Press.
Kohfeld, K.E., and Harrison, S.P., 2001. DIRTMAP: The geological record
of dust. Earth-Sci. Rev., 54,81–114.
Liao, H., and Seinfeld, J.H., 1998. Radiative forcing by mineral dust aero-
sols: Sensitivity to key variables. J. Geophys. Res., 103, 31637–31645.
Marticorena, B., and Bergametti, G., 1995. Modeling the atmospheric dust
cycle 1. Design of a soil-derived dust emission scheme. J. Geophys.
Res., 100, 16415–16430.
Prospero, J.M., Ginoux, P., and Torres, O., 2002. Environmental character-
ization of global sources of atmospheric soil dust identified with the
NIMBUS-7 TOMS absorbing aerosol product. Rev. Geophys.
doi:10.1029/ 2000RG000095.
Shao, Y., Raupach, M.R., and Findlater, P.A., 1993. Effect of saltation
bombardment on the entrainment of dust by wind. J. Geophys. Res.,
98, 12,719–12,726.
Sokolik, I.N., and Toon, O.B., 1999. Incorporation of mineralogical com-
position into models of the radiative properties of mineral aerosol from
UV to IR wavelengths. J. Geophys. Res., 104, 9423–9444.
Cross-references
Climate Forcing
Dust Transport, Quaternary
Eolian Dust, Marine Sediments
Eolian Sediments and Processes
Iron and Climate Change
ALBEDO FEEDBACKS
Technically, the term albedo refers to the reflecting power of a
body, or a surface area, and is the fraction of light reflected by
the body relative to the total light that is incident on the body,
or surface. Thus, a perfect absorber has an albedo of zero while
a perfect reflector has an albedo of unity. In general, the albedo
of a given surface is a function of wavelength. It is also depen-
dent on the angle at which the incident light strikes the reflect-
ing surface. This leads to more specialized terminology such
as “spectral albedo” and “plane albedo,” which refer to the
wavelength and solar zenith angle dependence of the reflected
radiation, respectively. Reflected radiation that has been aver-
aged over all possible angles of incidence is called “spherical
albedo.” It is also referred to as the Bond albedo, which is
the ratio of the total light reflected by a spherical body, such
as a planet, to the total radiation that is incident on the planet.
In the context of terrestrial climate where interest is primar-
ily directed toward describing the radiative energy balance of
the Earth, there is an implicit assumption that the spectral
albedo has been integrated over all wavelengths. The albedo
of the Earth is then directly related to the solar energy that is
available to drive atmospheric dynamics and for maintaining
the temperature of the Earth-atmosphere system. It is also useful
to differentiate between “surface albedo,” which is the reflectiv-
ity of the bare ground and ocean surface without including atmo-
spheric scattering effects, and “planetary albedo,” which includes
all atmospheric and surface scattering contributions and refers to
the outgoing solar radiation at the top of the atmosphere that is
reflected back to space.
Figure A1 shows the zonally averaged annual mean surface
albedo (dashed line) and planetary albedo (solid line) of the Earth
as computed with a general circulation climate model. Character-
istically, the ocean and land are areas of low reflectivity with typ-
ical albedos in the 6–12% range. Substantially higher surface
albedos due to snow and ice are evident in the polar regions
where the albedo of fresh snow may exceed 90%. Globally aver-
aged, the surface albedo of the Earth is about 12%. The planetary
albedo is generally larger than the surface albedo due primarily to
the high reflectivity of clouds. As in the case of fresh snow, the
albedo of optically thick clouds can sometimes exceed 90%.
However, because of absorption by atmospheric gases and multi-
ple scattering effects between the atmosphere and ground sur-
face, the planetary albedo in the polar regions is frequently
lower than that of the highly reflecting snow surface. Globally
averaged, the planetary albedo of the Earth, also called the global
albedo, is approximately 30%. Thus, about 70% of the solar
2 ALBEDO FEEDBACKS